01 Aug 2023
The advent of laser cooling revolutionized the field of atomic physics, transforming atomic clocks and GPS technology and laying the foundations for quantum computing with atoms and ions. In the first of a three-part series, Chad Orzel tells the story of its early years
The idea that you can reduce an object’s temperature simply by shining light on it is gloriously counterintuitive. It even surprises physicists. The first time atomic physicist Hal Metcalf heard about the concept we now know as “laser cooling”, he was sure that the speaker – a young PhD student by the name of Bill Phillips – was talking nonsense. “In my best professorial manner, I lectured him and said ‘Look, if you put energy into the system, it can’t cool’,” recalls Metcalf, now a Distinguished Teaching Professor at Stony Brook University in New York, US. “And he said ‘Sit down, Metcalf, you’ve got some stuff to learn.’”
Metcalf’s initial confusion is understandable. Although using energy to cool something is, of course, routine – it’s what happens in your refrigerator – there is something about the idea of using nothing but light to cool a gas of particles, to temperatures a fraction of a degree above absolute zero, that makes even experienced physicists scratch their heads. Temperature is a measure of the average energy of the particles in any system, and in physics as in popular culture, we usually think of lasers as a tool for adding energy to a system: raising electrons to a higher orbit, or blasting a spaceship into vapour in a sci-fi movie. It seems inconceivable that shining concentrated light on a gas could instead remove some of its energy.
With a few Nobel prizes’ worth of care and ingenuity, though, laser cooling is very much possible, and its rewards have been substantial. In the nearly 50 years since Metcalf tried to convince Phillips that it wouldn’t work, laser cooling has revolutionized the fields of atomic, molecular and optical physics. Laser cooling of atoms and ions has enabled dramatic leaps in the precision of atomic clocks, allowing new tests of fundamental physics and potential improvements in clock-based navigation via the Global Positioning System (GPS). It has opened new avenues in the study of quantum-mechanical phenomena like superfluidity and superconductivity, helping us explore regimes that can’t readily be accessed with conventional materials.
More recently, it has provided one of the best current platforms for the study of quantum information and quantum computing, now one of the hottest fields in technology, which has attracted billions in funding from government agencies and major corporations. Cooling atoms to within millionths or billionths of a degree above absolute zero allows their quantum nature and properties to be measured with unprecedented precision. All of this has put atomic and molecular physics on the cutting edge of quantum science.
As usual, everything goes back to Einstein
The essential physics behind laser cooling can be traced back to Albert Einstein’s 1917 observation that photons, the fundamental “particles” of light, must carry momentum, and this momentum can be used to change the motion of atoms. In 1933 Otto Frisch demonstrated this momentum transfer in a tour de force experiment that used light from a sodium-vapour lamp to deflect a beam of sodium atoms. The degree of deflection (around 1 mm) was tiny, however, so the idea of using light to push atoms around remained a curiosity. This remained true until 1960, when the invention of the laser introduced a light source bright enough to generate substantial forces.READ MORE

The study of manipulating matter with light began in earnest with Arthur Ashkin, a physicist at Bell Labs during its late-1960s heyday as a centre of innovation and basic research. Back then, the concept of a telecommunications company paying someone to study light forces on atoms was not as outlandish as it might be today. “Basically, the instruction we were given was ‘You can do whatever you want to do, but it’s got to be world class,’” recalls Ashkin’s then-colleague John Bjorkholm, who went on to do his own world-class research on the ultraviolet lithography techniques used to make state-of-the-art computer chips.
Thanks to some back-of-the-envelope calculations, Ashkin realized that the forces from laser photons bouncing off a microscopic object could be significant. Encouraged, he began experimenting with an argon laser and polystyrene beads in water. As predicted, the laser pushed the beads through the water and pinned them against the downstream window of their container (figure 1).
1 Light forces on atoms
(a) The scattering force arises when an atom absorbs an incoming photon of light, giving the atom a “kick” that starts it moving in the direction of the photon. Re-emitting the light also gives the atom a kick, but in a random direction. Over many cycles, these random kicks cancel out and the net effect is a force in the direction of the light. (b) The “dipole force” arises from an interaction with the light that lowers the energy of the atom’s ground state, with the shift being largest in the centre of a beam where the intensity of the light is highest. The atom experiences this as a force pulling it into the high-intensity centre of the beam.
Ashkin published his first results in 1970, demonstrating that lasers could both accelerate beads and trap them in a region between two laser beams propagating in opposite directions. In subsequent years, he and his Bell Labs colleagues went on to demonstrate the levitation of small objects with vertical light beams and the trapping of small objects in the centre of a tightly focused laser beam. The latter technique allows particles to be manipulated by moving the focus point, and is now known as “optical tweezing”. It has since found numerous applications in biology, and in 2018 Ashkin was awarded half of the Nobel Prize for Physics “for the optical tweezers and their application to biological systems”.
From tweezers to coolers
Ashkin’s work launched the study of light forces on microscopic objects, but for smaller chunks of matter, the baton passed to two other groups: one led by Theodor Hänsch and Arthur Schawlow at Stanford University (each would go on to win their own Nobel prizes for work on laser spectroscopy, Schawlow in 1981 and Hänsch in 2005), and another by Hans Dehmelt and Dave Wineland at the University of Washington. Both groups’ papers appeared in 1975 (the latter as an abstract for a conference presentation, due to Dehmelt’s bad experiences with reviewers), and they considered situations that were superficially very different. While Hänsch and Schawlow pondered the effects of lasers on neutral atoms, Wineland and Dehmelt focused on ions in an electromagnetic trap.
Either way, the key physics was the same. If a laser is tuned to a frequency of light just below the frequency absorbed by a particular particle (“red detuned”), stationary atoms or ions will not absorb its light. When the particles are moving “upstream” against the light, however, they “see” the frequency of the light Doppler-shifted upwards, and become more likely to absorb a photon. When that absorption happens, the resulting momentum “kick” is necessarily in the opposite direction to the atom or ion’s velocity, so the particle slows down (figure 2). The net effect is that the laser only slows things down; it never speeds them up. This reduction in velocity corresponds to a reduction in temperature, which is a measure of the average energy of the atoms in a gas.
2 Light forces and Doppler cooling
(a) An atom at rest can absorb a laser photon whose frequency is tuned to match the energy difference between two states of one of its electrons. In this case, the atom also acquires the photon’s momentum, giving it a “kick” so it begins moving in the direction of the photon. (b) When the laser frequency is tuned below that associated with the energy difference, the atom will not absorb the photon, and does not change its motion. (c) If the laser frequency is too low to be absorbed, but the atom is moving in the opposite direction, the atom will see the light Doppler-shifted up (blue arrow) to the correct frequency, and will absorb it. The resulting momentum kick reduces the speed of the atom, producing a cooling force.
The first complete description of the cooling force, including a derivation of what would come to be known as the minimum achievable temperature or “Doppler cooling limit”, was published in 1977 by a trio of Soviet scientists (Vladilen Letokhov, Vladimir Minogin, and Boris D Pavlik Optics Comms 19 72). Numerous other calculations followed, all suggesting a lower limit of a millikelvin or a few hundred microkelvin above absolute zero. This expectation would shape the goals for the community for the better part of the following decade. And as we will see in part 2 of this history, it would prove to be stunningly wrong.
Laser whisperers and ion trappers
By the end of 1975, Wineland had moved on to Boulder, Colorado, and a staff position at what was then the National Bureau of Standards (it was renamed the National Institute of Standards and Technology, or NIST, in 1988). The new job came with generous research funding and a great deal of freedom. Though Wineland’s main duty was to support and evaluate the caesium atomic beam clock (then the primary frequency standard for the US), he recalls that “the guy that hired me wanted to try these new things, like laser cooling”.
With his boss’s blessing and some funding from the Office of Naval Research, Wineland began an experiment to laser-cool trapped ions. This required three elements: the ion traps he knew from his work with Dehmelt; a vacuum system to contain the trap and ensure that only ions from the element of interest were present (since the cooling mechanism depends on the characteristic frequency of the atoms, no other element will be affected by the light); and a laser system to cool the trapped ions. By his own admission, Wineland “knew nothing about lasers”, so he recruited a NIST chemist, Bob Drullinger, to be the experiment’s “laser jock”. “At that time lasers were really primitive,” Drullinger says, “and it didn’t take a lot of skill to be considered a laser whisperer.”
Ion trappers Wayne Itano, Jim Bergquist, Dave Wineland and Bob Drullinger of the National Bureau of Standards (later NIST) Ions Group in 1979. (Courtesy: David Wineland/National Institute of Standards and Technology. Used with permission. Rights reserved.)

Drullinger put together a system that could produce enough ultraviolet light to demonstrate laser cooling on a sample of magnesium ions. This is not a simple process even today, and in 1977 it was a major challenge. According to Drullinger, it took around six weeks of intensive effort, starting from an empty room and a lot of equipment in crates, before he and Wineland were ready to make their first attempt at cooling ions.
The initial experiment used a cloud of around 50,000 trapped magnesium ions. To monitor the ions’ behaviour, Drullinger and Wineland measured the currents the ions’ random motion induced in the trap electrodes. The faster the ions moved, the bigger the currents they created, so the signal they produced was essentially random noise at an overall level that depended on the temperature of the ion cloud.
The first night the apparatus came together, Drullinger recalls that it was nearly midnight before they had everything ready. As we’ll see again with the cooling of neutral atoms, this is fairly typical for such experiments. Wineland and Drullinger fired up the trap, turned on the laser and immediately saw the cooling signature they expected. “We did the first scan, and bang! There it was,” Drullinger recalls. The noise from thermal motion dropped dramatically when illuminated with a red-detuned laser, down to a level so low they could not even estimate the ions’ temperature. When they changed the laser frequency to make it blue-detuned, the trapped ions heated up, exactly as expected. It was a great success.
From delight to disappointment, and back
Of course, it wasn’t quite as simple as that. After taking preliminary measurements, Wineland and Drullinger called it a night. The next day, the signal was much worse; the laser had an effect, but not nearly as dramatic. On the third night, they saw nothing. While the electronic signal indicated ions were trapped, the laser didn’t seem to be doing anything at all.
Baffled and disappointed, they tore down their apparatus and rebuilt it. Eventually, they discovered the problem: they had exhausted their supply of magnesium. In fact, in their efforts to continue trapping ions, they had pushed the temperature of their failing magnesium source (commonly referred to as the “oven” in this field) so high that sodium ions began boiling out of the glass port of the vacuum chamber. Sodium and magnesium have nearly the same atomic mass, so the trapped-ion signal looked similar, but they absorb light at very different frequencies, so it was no surprise that Wineland and Drullinger weren’t cooling anything. They duly reloaded the source with magnesium, and once they resumed trapping the correct element, the cooling signal returned.
Wineland and Drullinger wrote up their results and submitted them to Physical Review Letters (40 1639). Curiously enough, a rival group in Heidelberg, Germany was also doing laser-cooling experiments (aided by a visiting Dehmelt), and the two groups’ papers reached the journal one day apart: first the Heidelberg team’s paper on barium ions, then the Boulder trio’s on magnesium. But as luck would have it, the editing process for the Heidelberg paper (Phys. Rev. Lett. 41 233) took longer, so Wineland, Drullinger and Walls’ was the first to print, in June 1978.
I turned to Dave and said ‘Where do we go from here?’ Very slowly, a little grin spread across his face, and he said, ‘Stockholm’Bob Drullinger
Sometimes, it takes a while for the full significance of a discovery to become clear. This time, it was pretty much immediate. On that first late night, Drullinger recalls that he and Wineland spent some time “sitting there just enjoying the moment. We’re on opposite sides of the table, and the room was dark, just the glow of the lasers. I was eager to get on to the next step, but I couldn’t immediately figure out what that was. So I turned to Dave and I said ‘Where do we go from here?’ Very slowly, a little grin spread across his face, and he said, ‘Stockholm’.”
Wineland was right. In 2012 he shared the Nobel Prize for Physics “for ground-breaking experimental methods that enable measuring and manipulation of individual quantum systems”.
The problems of neutral atoms
Wineland’s prize was not, however, the first Nobel to be awarded to a NIST physicist for laser cooling. That honour went to work that happened chronologically later, in the mid-1980s, on the opposite side of the US. At the same time as Wineland’s group was getting off the ground in Boulder, Bill Phillips was finishing his PhD at the Massachusetts Institute of Technology (MIT). At the celebration following Phillips’ defence, his supervisor joked that it was good that he had done a side project on lasers during his PhD, because if his only work was his original project on magnetic resonance, he would’ve ended up “stuck at the National Bureau of Standards”.
Phillips did, in fact, end up at the National Bureau of Standards, but in Gaithersburg, Maryland, rather than Boulder, Colorado. Like Wineland, he was hired to contribute to a core project – an improved measurement of the ampere, the unit of electric current – with an agreement that he could spend some time, in his words, “fooling around with atomic physics”. And so he set up a small lab for laser cooling sodium atoms.
It’s a trap Arthur Ashkin in 1971 with his lab apparatus capable of levitating small particles in an intense laser beam. (Courtesy: Nokia Bell Labs, Nokia Corporation and AT&T Archives)
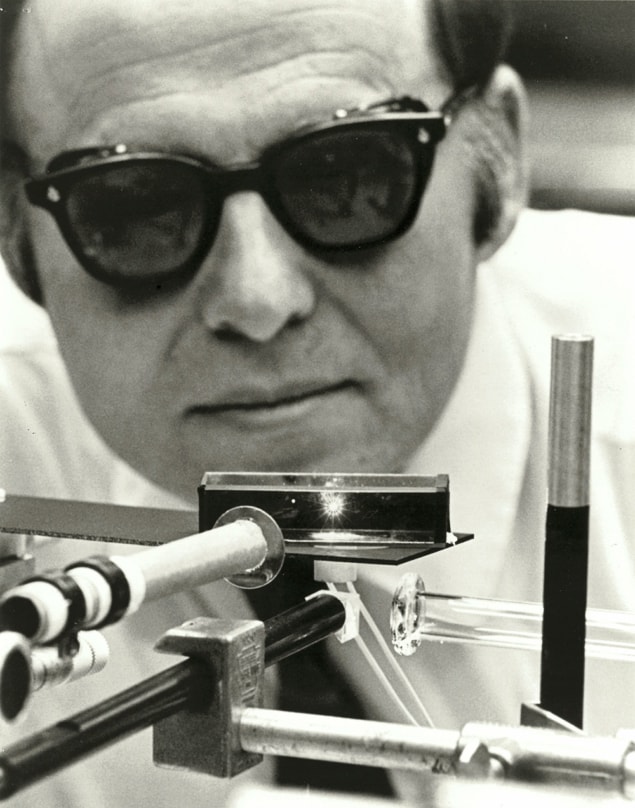
Phillips’ inspiration was a 1978 paper in which Ashkin proposed trapping neutral atoms between two tightly focused laser beams (Phys. Rev. Lett. 40 729). Neutral atoms are much more difficult to trap than ions, whose electrical charge means they experience large forces from magnetic fields or high-voltage electrodes. In the absence of an electric charge, the only forces that can easily be applied to atoms come from the scattering force (momentum transfer when photons are absorbed) and the dipole force (which lowers the atom’s internal energy in the presence of light, and thus pulls it into the centre of a beam). Both of these are relatively tiny. Making an optical trap strong enough to catch fast-moving atoms from a hot vapour is thus a tricky business.
Still, Phillips was struck by the idea. He even explored it briefly while a postdoc at MIT, only to conclude that the problem was more complicated than he initially thought, and best filed away for when he had his own lab. His trial run encountered two problems with the prevailing simple picture of laser cooling. One of these is the same Doppler shift that makes cooling possible. A sodium atomic beam emerges from an oven at speeds approaching 1000 m/s. This produces an enormous Doppler shift in the frequency of the light needed to slow the beam. But as the atoms absorb photons and slow down, the Doppler shift decreases and the cooling becomes less effective. After a reduction of just a few metres per second, the atoms quit absorbing the light and the slowing stops.
The second problem is that the Doppler cooling concept envisions atoms with only two energy levels: a ground state and an excited state. But real atoms have multiple low-energy states, and only one of them can absorb light from the laser. Hence, after a handful of cycles of photon absorption and re-emission, atoms tend to decay into a “dark” state and stop interacting with the laser.
One really good idea
By the time Phillips was ready to try cooling atoms again, other research groups had identified some potential fixes. The multiple-states problem proved relatively straightforward to solve: adding a second “repumper” laser to the experiment returns atoms from the dark state back to the bright one so that slowing can resume. Fixing the Doppler shift was trickier. Possible solutions included using a broad range of frequencies in the slowing beam (“white-light cooling”) and sweeping the frequency of the cooling laser to follow the changing Doppler shift (“chirp cooling”), but both of these methods require expensive and complicated modifications to the laser source.
After consulting with Metcalf, Phillips opted for a third approach, one that he describes as “the only good idea I’ve ever had”. Rather than adjusting the frequency of the laser to match the changing velocity of the atoms, he used a magnetic field to adjust the frequency of the atoms to match that of the laser. This is possible because of the Zeeman effect: a neutral atom placed in a magnetic field sees its energy levels shift up or down by an amount that depends on the strength of the field (figure 3).
3 Zeeman slowing
Fast-moving atoms in the atomic beam enter a tapered electromagnet at the right, and have their energy levels shifted by a large magnetic field (dashed blue arrow), which compensates for the large Doppler shift (light blue arrow), allowing them to absorb photons from the laser. At the left end, the field has decreased significantly, but so has the Doppler shift, allowing the same laser to continue to interact with the atoms.
Thanks to this Zeeman shift, a tapered magnetic field can compensate for changes in the Doppler shift as the atoms slow down. Close to the oven, where the atoms are moving very fast and have large Doppler shifts, the field is very large, leading to a large energy shift. At the far end, where the atoms are moving slowly, the field is much smaller, and both the Doppler and Zeeman shifts are small. With the right choice of fields, then, the same laser beam interacts with both sets of atoms, extending the cooling process.
Phillips’ masterstroke had one further benefit. As well as compensating for the Doppler shift, the magnetic field of the so-called Zeeman slower shifts the atoms’ energy levels in a way that almost completely eliminates the need for repumping. A single laser, shining down the bore of a tapered electromagnet, can bring a beam of sodium atoms to a near-complete stop using only scattered light. “Everything else was just luck,” Phillips reflects, “but [Zeeman slowing] was actually a good idea.”
Catching atoms in a magnetic trap
Phillips and Metcalf next turned their attention to trapping the atoms. Using two coils of wire placed inside the vacuum chamber that keeps the atoms isolated from their environment, they created a magnetic field with a minimum in its centre. The result is, again, a Zeeman shift, but this time it weakly traps the atoms as well as slowing them. In order for an atom to move out from the centre, it has to increase the energy of its orbiting electron, and the energy to do that must come out of its motion, slowing it down. Eventually, it comes to a stop and reverses direction, back toward the centre of the trap.
Much like Wineland and Drullinger a few years earlier, Phillips and Metcalf made their first attempt at magnetic trapping late at night. “Everything always happens late at night,” says Phillips, “because if you could have gotten everything working before late at night, you would’ve done the experiment already, but you have to learn how to get everything working first.”
Suspense Bill Phillips working on laser-cooling experiments in his laboratory circa 1986. (Courtesy: Phillips/National Institute of Standards and Technology. Used with permission. Rights reserved.)

In this case, each experimental run consisted of turning on the laser for the fraction of a second needed to slow atoms, then turning the laser off and the magnetic trap on, then waiting an additional period of tens of milliseconds before flashing another laser pulse on to look for fluorescence emitted by atoms held in the trap. The sequence of laser pulses was controlled by a rotating cardboard disc with holes cut in the appropriate places – “These were really prehistoric times,” Phillips jokes – and after a long day, they finally had all the timings right.
Unfortunately, they didn’t see any atoms. “So we said, ‘Okay, let’s get something to eat,’” Phillips recalls. When they returned, they turned the system back on. Immediately, everything worked. Over the course of a very long day, the high current running through the magnetic-trap coils had gradually heated the coils enough to degrade the vacuum inside the chamber. The slow sodium atoms that should’ve been trapped were thus knocked out by collisions with unrelated “background” atoms boiled out of the coils. But during Phillips and Metcalf’s late-night fast-food break, the coils cooled down and the vacuum recovered, so everything worked as designed.
Wahoo This chart, from 15 March 1985, indicated to Bill Phillips and his fellow researchers that they had succeeded in capturing atoms with their magnetic trap. Group member Hal Metcalf labelled the good news with “WAHOO!!” (Courtesy: Bill Phillips/National Institute of Standards and Technology. Used with permission. Rights reserved.)
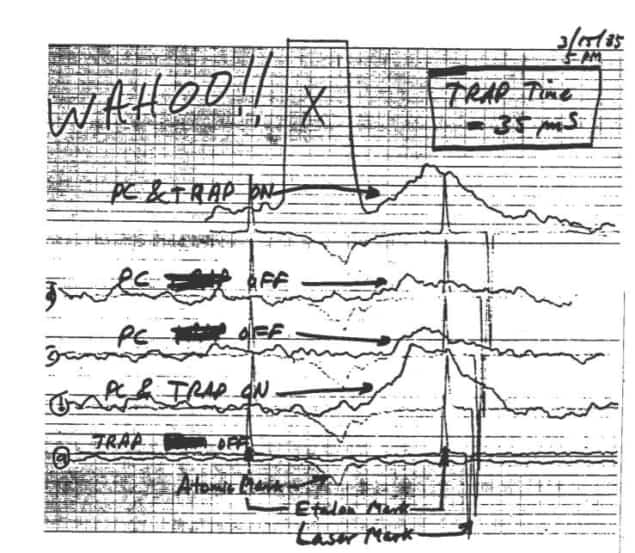
Buoyed by this success, Metcalf scrawled “Wahoo!” on the chart recorder trace showing the fluorescence signal that confirmed the magnetic trap was holding on to atoms loaded from the slower. The pair then worked through the night. At sunrise, they returned to Phillips’s house. “We hunted around in the fridge and found some ice cream,” Metcalf recalls, “And [Phillips’ wife] Jane came down and said, ‘That ice cream is for the kids! What are you guys doing?’ And so we told her, ‘Well, we had a pretty good night, we’re celebrating.’”
They had earned the ice cream. Phillips and Metcalf’s paper on the first magnetic trapping of neutral atoms was published in 1985 (Phys. Rev. Lett. 54 2496). A little over a decade later, in 1997, it helped to secure Phillips a share of the Nobel Prize for Physics “for development of methods to cool and trap atoms with laser light”.
A tale of three Nobels
The early years of laser cooling are thus a tale of three Nobel prizes: Ashkin’s for proving it was possible to manipulate small objects with light; Wineland’s for extending the principle to ions; and Phillips’ for showing that atoms, too, could be trapped and tamed with beams of photons. But in the mid-1980s, new researchers joined the burgeoning field of laser cooling, bringing new technologies and techniques for cooling and trapping, some of which would turn out to work better than theory said was possible. The process of revolutionizing atomic, molecular and optical physics was just getting started.Part 2 of the history of laser cooling by Chad Orzel will be published soon on Physics World
Chad Orzel is a popular-science author and chair of the Department of Physics and Astronomy at Union College in the US. He has a PhD in chemical physics from the University of Maryland, College Park, where he studied laser cooling at the National Institute of Standards and Technology in the lab of Bill Phillips, who shared the 1997 Nobel Prize for Physics
from physicsworld.com 11/8/2023
Δεν υπάρχουν σχόλια:
Δημοσίευση σχολίου